John M. Willis, Master’s Student, Sustainable Development, University of Sussex
Abstract
The current climate emergency calls for an “all-hands-on-deck” approach to transforming many aspects of our lives in order to reduce and reverse global warming. Homes and the energy consumed in daily living are a non-trivial contributor to climate change. A current technological trajectory is to use solar photovoltaic panels and batteries to address the problem. However, they both use toxic substances in their production, have critical material supply concerns, have relatively short productive lifetimes, and lack proven plans for recycling. The ways in which home building envelopes are constructed today are very inefficient compared to the Passive House standard. If this standard were applied to the construction of every new single-family home, the energy required for the operation of all new homes would be drastically reduced. Then, the use of solar and ground sourced thermal energy using existing technologies may provide the heating, cooling, and electrical energy needed without the use of photovoltaic panels. Thermal energy storage, coupled with compressed air energy storage, may provide long-term energy storage without the need for batteries. Existing literature on these lower ecological footprint technologies will be reviewed through a thermodynamics systems lens.
How to cite this poster:
Willis, John. Transforming energy performance of new homes using a thermodynamics systems paradigm. Tipping Points – From Climate Crisis to Positive Transformation, University of Exeter, United Kingdom, September 2022. Unpublished conference paper. Print. Available at: https://www.johnmwillis.me/wp-content/uploads/2022/09/Transforming-energy-performance-of-new-homes-using-a-thermodynamics-systems-paradigm-John-M.-Willis-Tipping-Points-Conference-2022-Univ-of-Exeter-UK.pdf.
Introduction
This poster will cover three key points. First, that the current paradigm of using primarily solar photovoltaic panels and batteries in modern construction is not sustainable. Second, a paradigm leveraging the thermodynamic properties of key renewable energy system components is proposed. Third, the potential impact of widespread adoption of the paradigm on climate change targets will be discussed.
The Current Paradigm is Not Sustainable
Two key aspects to the current paradigm include energy intensity required by “modern” home construction practices, and the sustainability of the materials used for energy system components.
The energy demands of current buildings far exceed what is needed compared to demand when Passive House standards are applied to the building envelope. The typical Energy Performance Index of buildings in various countries is contrasted in Figure 1.
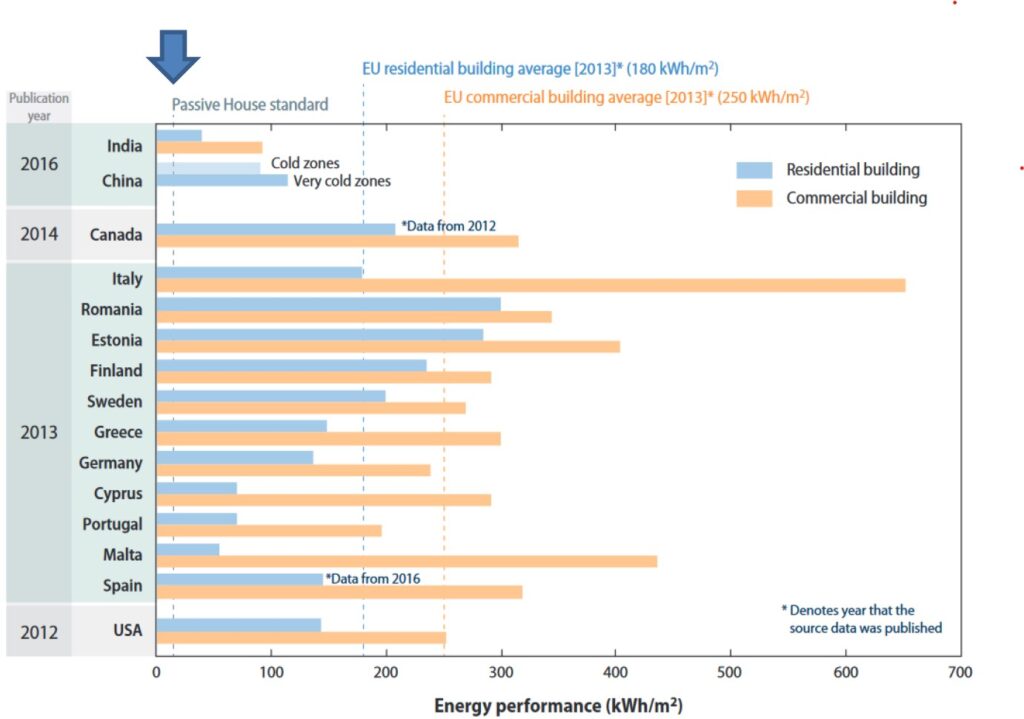
Figure 1. Energy performance indexes for residential and commercial buildings (Ürge-Vorsatz et al., 2020)
With an annualized 916,000 new single-family homes built in the United States (‘United States Housing Starts’, 2022), the opportunity to avoid excessive energy demand by applying Passive House standards is rather significant.
Key quotes from relevant literature regarding environmental and supply issues with solar photovoltaic panels and batteries include:
- The increase in PV panel waste is anticipated to reach over 60 million tonnes by 2050, mostly disposed of in landfills where groundwater and soil contamination may occur (Franco and Groesser, 2021, p. 1). “A similar growth/waste scenario is expected for lithium-ion batteries” (ibid., p 22).
- “Claims about the sustainability of PV [photovoltaic solar panel] technologies cannot be fully supported until efficient and environmentally-friendly recycling processes for them have been developed and are deployable” (Ardente et al., 2019, p. 166).
- “To be comparable with renewable energy systems, hydroelectric, wind, biomass, geothermal and solar (4–76 g CO2 eq kWh−1), 300 folds reduction in the GWP [global warming potential] of BESS [battery energy storage systems] will be necessary” Sadhukhan and Christensen (2021).
- The future availability of critical raw materials for solar PV panels is in question (Cristobal et al., 2020), so much so that some researchers are investigating extraterrestrial bodies as another source (Dallas et al., 2021).
Reducing the building energy intensity required by nearly an order of magnitude through the use of Passive House standards enables the use of approaches to renewable energy systems design that were not previously economical. In addition, it provides the opportunity to revisit the paradigm applicable to their design.
Hypothesis
For new builds of single-family homes using the Passive House standard it is practical to reduce energy demands to the point where it is possible to apply thermodynamic principles to the design of renewable energy systems which would not require the use of photovoltaic panels nor batteries. Figure 2 provides a simplified conceptual perspective.
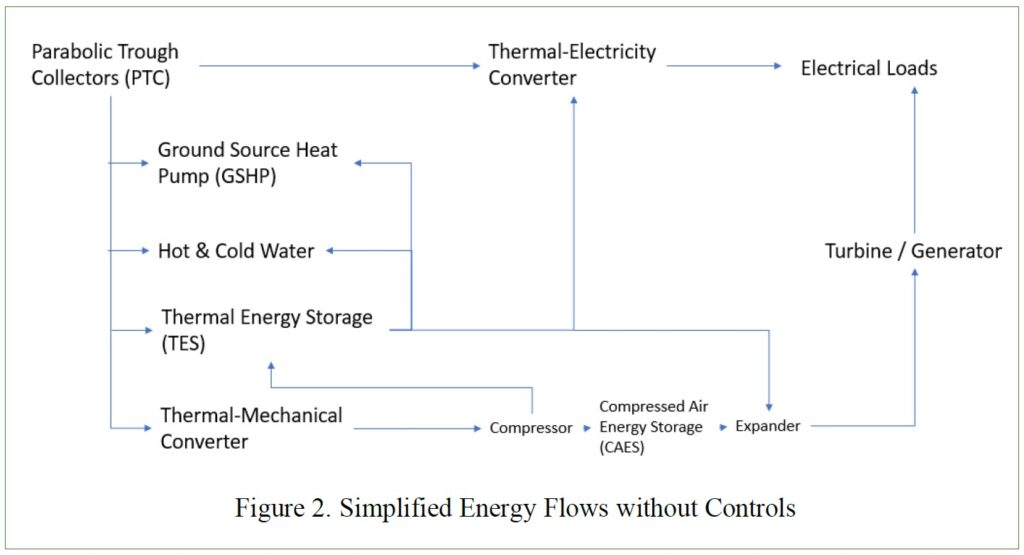
The Role of Thermodynamics in a Renewable Energy System
The research led to considering the following key components:
- Solar thermal collectors (parabolic trough collectors)
- Ground Source Heat Pumps
- Thermal Energy Storage (TES)
- Stirling and Organic Rankine Cycle Engines (for energy conversion)
- Compressed Air Energy Storage (CAES), plus expander and turbine/generator
When implementing these, there are numerous ways to select and configure them as a system. Key principles to keep in mind include:
- The sun is the primary source of heat (and is variable and intermittent).
- The ground is a primary source of cold (more or less constant).
- The air can be a source of hot or cold and is variable.
- Temperature sources are best used for temperature-based applications.
- Hot and cold temperature sources should both be stored separately.
- Hot and cold sources may be shared to heat or cool air and water.
- Hot and cold sources may be added together in their storage, or at the time of use.
- Heat is the primary source of electricity by way of Stirling or ORC engine & generator/inverter.
- Hot and cold sources may include energy system components themselves.
- Certain functions may not yet be economically feasible using a thermodynamics approach.
- Thermal energy may be converted to mechanical energy using a Stirling or ORC engine.
- Compressed Air Energy Storage persists longer than Thermal Energy Storage.
- CAES is used for generating electricity only after TES has dropped below a certain level.
- Solar collectors and compressed air tanks require sufficient physical space.
Highlights of Key Component Characteristics
Stirling Engines to Convert from Heat to Electricity
A number of solar power implementations use Stirling or Organic Rankine Cycle (ORC) engines to directly couple to solar collectors to convert thermal energy to electricity. A variety of systems in the 1 to 3.2 kWe range are commercially available, have as high as 24% net system efficiency and operate on as little as 725 W/m2 Direct Normal Irradiance (DNI) (Giovannelli, 2015, p. 610). Most notable is the EU Project DiGeSPo, using parabolic trough collectors (PTC) and a Stirling engine, that demonstrated 3 kWe output with 12-15% electrical efficiency using a 250-350°C temperature source (ibid.; Crema et al., 2014, p. 32). Using numbers from Orosz et al. (2009), with 1,130 sq. ft. of PTC array area, an ORC system cost of $14,400 yielded a 3 kWe system.
Thermal Energy Storage (TES)
One study used readily available HVAC components for a 2.8kWe PTC-ORC system with pebble-bed
thermal energy storage (TES) (Dickes et al., 2014). Typical costs of building scale TES systems are around $30 USD /kW hth, making them cost-competitive with battery energy storage systems (Odukomaiya et al., 2021, p. 5321). Storage timeframes for TES systems are typically around 12 hours.
Ground Source Heat Pumps, Plus Solar Assistance
Ground Source Heat Pumps (GSHPs) are not installed as often as Air Source Heat Pumps (ASHPs) because of the cost of excavation. However, GSHPs have a payback period of 5-7 years (Turpin, 2014), 30-80% savings over conventional approaches, including ASHPs, and average at least 50% less greenhouse gas (GHG) emissions than ASHPs (Aquino et al., 2021). An indirect expansion solar assisted GSHP in parallel mode was able to attain an overall coefficient of performance (COP) of 3.96 (Nouri, Noorollahi and Yousefi, 2019, pp. 227, 229). ‘2022 Geothermal Heat Pump Cost & Heating System Installation Prices’ (2022) suggests that excavation, equipment and installation of a standard GSHP is $4,000 to $8,000 USD per ton.
Ground Source Heat Pump with Thermal Storage
Pairing GSHPs with thermal energy storage (TES) using salt hydrate Phase-Change Materials (PCM) could reduce the cost of excavation by 50%, and decrease energy consumption between 12.8 to 32%, while achieving a coefficient of performance (COP) of 2.0 to 6.49 (Aquino et al., 2021; Zhu et al., 2014, p. 150). According to the author’s calculations, it appears that for the same cost as a standard GSHP, it is possible to include the TES at no additional cost.
Converting Thermal Energy into Compressed Air Energy Storage (CAES) for Later Electricity Generation
The act of compressing air results in heat that should be stored. Upon expansion, the heat may be retrieved and added back to the air. This is known as adiabatic CAES. It is not straightforward to use thermal energy from solar collectors coupled to a Stirling or ORC engine to mechanically compress air into a storage tank because of the increasing pressure in the tank, although multistage Stirling engine based thermocompressors are in the early stage of development (Fischer and Kuehl, 2021). CAES is capable of storing energy longer than TES and batteries.
A few studies have focused on small-scale CAES using compressed air tanks. Rukh and Khattak (2020) studied the discharge power characteristics for a system rated at 4kW. Castellani et al. (2018) demonstrated that at an operating pressure of 225 bar stored in a volume of 0.25 m3, it was possible to produce 1.273 kWh, covering 26% of the 4.6kW energy demand (i.e., during times of low or no sun, or high demand). Although CAES is not as efficient in charging as batteries, batteries have a lifetime of about five years, while a CAES has lifetime exceeding 20 years—suggesting that a Life Cycle Assessment (LCA) comparing the two is needed (Castellani et al., 2018). Maia et al. (2016, p. 359) suggests that a 10 kW CAES turbine generator system may be constructed for under $5,000.
Discussion
Assuming a 2,200 sq. ft. home in central Pennsylvania in the U.S. built to Passive House standards, typical GSHP COP of 3.89 and improved COP of 6.49 using TES, only a 1-ton GSHP would be required based on the author’s calculations. Considering the cost of modern home with a solar photovoltaic and battery solution today, use of the Passive House standard and components described above may eliminate or minimize the need for PV panels, batteries, and grid-tie—at an equal or lower cost.
Assuming 10 million net new homes being built per year globally to meet population growth, with a current typical 8 tons of CO2 being emitted per home under “modern” building practices, adoption of a Passive House building standard using an integrated thermodynamics systems approach may avoid adding 80 million tons of CO2 per year at a time when we are trying to reduce emissions each year.
Conclusion
This poster covered three key points:
- The current paradigm of adding solar photovoltaic panels and batteries to construction that does not minimize operating energy intensity is not sustainable.
- A thermodynamic systems paradigm was proposed to leverage the properties of key renewable energy system components, and is cost-competitive.
- The potential impact of widespread adoption of the paradigm on climate change targets is significant.
Further research is required to model the various components and ways to connect them together. Certain components may not be available at the desired scale. Other components may need to be designed, or re-designed to support efficient integration. There are numerous opportunities for integrating these technologies to achieve a multiplier effect of increased efficiencies.
References
‘2022 Geothermal Heat Pump Cost & Heating System Installation Prices’ (2022) HomeGuide. Available at: https://homeguide.com/costs/geothermal-heat-pump-cost (Accessed: 5 September 2022).
Aquino, A., Scrucca, F. and Bonamente, E. (2021) ‘Sustainability of Shallow Geothermal Energy for Building Air-Conditioning’, Energies (Basel), 14(21), p. 7058.
Ardente, F., Latunussa, C.E.L. and Blengini, G.A. (2019) ‘Resource efficient recovery of critical and precious metals from waste silicon PV panel recycling’, Waste Management, 91, pp. 156–167.
Castellani, B. et al. (2018) ‘Small-Scale Compressed Air Energy Storage Application for Renewable Energy Integration in a Listed Building’, Energies, 11(7).
Crema, L. et al. (2014) ‘Distributed m-CHP generation from a small scale concentrated solar power installation’, University of Malta. Institute for Sustainable Energy.
Cristóbal, J. et al. (2020) ‘Life cycle losses of critical raw materials from solar and wind energy technologies and their role in the future material availability’, Resources, Conservation and Recycling, 161, p. 104916.
Dallas, J.A. et al. (2021) ‘Investigating extraterrestrial bodies as a source of critical minerals for renewable energy technology’, Acta Astronautica, 186, pp. 74–86.
Dickes, R. et al. (2014) ‘Experimental investigation of an ORC system for a micro-solar power plant’.
Fischer, F. and Kuehl, H.-D. (2021) ‘Analytical model for an overdriven free-displacer thermocompressor’, Applied Thermal Engineering, 185, p. 116251.
Franco, M.A. and Groesser, S.N. (2021) ‘A Systematic Literature Review of the Solar Photovoltaic Value Chain for a Circular Economy’, Sustainability, 13(17).
Giovannelli, A. (2015) ‘State of the Art on Small-Scale Concentrated Solar Power Plants’, 70th Conference of the Italian Thermal Machines Engineering Association, ATI2015, 82, pp. 607–614.
Maia, T.A.C. et al. (2016) ‘Experimental performance of a low cost micro-CAES generation system’, Applied Energy, 182, pp. 358–364.
Nouri, G., Noorollahi, Y. and Yousefi, H. (2019) ‘Designing and optimization of solar assisted ground source heat pump system to supply heating, cooling and hot water demands’, Geothermics, 82, pp. 212–231.
Odukomaiya, A. et al. (2021) ‘Addressing energy storage needs at lower cost via on-site thermal energy storage in buildings’, Energy & Environmental Science, 14(10), pp. 5315–5329.
Orosz, M. et al. (2009) ‘Small scale solar ORC system for distributed power’, in Conference SolarPaces 2009.
Rukh, G. and Khattak, A. (2020) ‘Development of a Prototype Uninterrupted Electrical Power Supply System using Compressed Air Storage from Renewable Energy Resources’, Mehran University Research Journal of Engineering and Technology, 39(2), pp. 237–246.
Sadhukhan, J. and Christensen, M. (2021) ‘An In-Depth Life Cycle Assessment (LCA) of Lithium-Ion Battery for Climate Impact Mitigation Strategies’, Energies, 14(17).
Turpin, J.R. (2014) ‘Touting the Benefits of Residential Geothermal HVAC Systems’, Air Conditioning, Heating & Refrigeration News, 252(13), p. 14.
‘United States Housing Starts’ (2022) Trading Economics. Available at: https://tradingeconomics.com/united-states/housing-starts (Accessed: 5 September 2022).
Ürge-Vorsatz, D. et al. (2020) ‘Advances Toward a Net-Zero Global Building Sector’, Annual Review of Environment and Resources, 45(1), pp. 227–269.
Zhu, N. et al. (2014) ‘Recent research and applications of ground source heat pump integrated with thermal energy storage systems: A review’, Applied thermal engineering, 71(1), pp. 142–151.
Acknowledgements
The research for this poster was conducted alongside, and as part of, online coursework related to the author’s Master’s studies at the University of Sussex, as well as the course Circular Economy and Sustainability Strategies at the Judge Business School, University of Cambridge, and the Buildings as Sustainable Energy Systems Professional Certificate program at the Delft University of Technology (TU Delft). Funding provided by ZeroNetEnergyHomes.com, a project of Turnaround Security, Inc.
Contact
For more information contact: SustDev.CSO@johnmwillis.me.